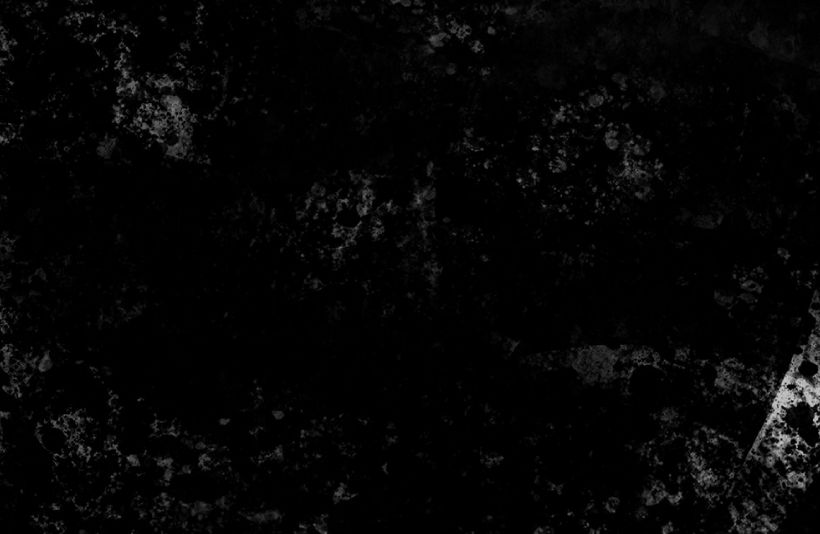
Rockets and Missiles
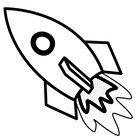
The principle of rocket propulsion has been known for nearly 2,000 years. In the Far East, rockets were used in warfare as early as the 13th century. Military interest in rockets declined in the 19th Century as developments in gunnery were superior to rockets in range and accuracy. In modern times however, guided missiles are at the forefront of any military action. As one of the more recent applications of projectiles, this section looks at the physics and applications of rockets and missiles.
Information in these green boxes is considered advanced reading! Read this information if you want to learn more.
A rocket is defined as a cylindrical projectile that can be propelled to a great height or distance by the combustion of its contents. The simplest rockets are just that, cylindrical tubes with fuel packed inside of them which generates thrust when made to combust.
​
A missile is any object that can be projected or thrown at a target. But in modern military usage, the word is synonymous with guided missiles. It is guided missiles which will be referring to here.
​
Developments in Rocket and missile technology can be divided roughly into three stages;
-
Early Rocket Technology: A quantity of propellant packed in a cylindrical tube.
-
Late Rocket Technology: Turbo-jets, pulse-jets, and ram-jets (all variations of jet engines which depend upon the presence of air for combustion of fuel)
-
Modern Missile Technology: Carry their own source of oxygen for combustion with fuel (not dependent on the presence of air)
Forces Acting on a Missile in Flight
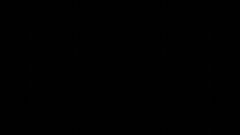
FORCES ACTING ON MISSILE DURING FLIGHT
To consider the physical principles behind a missile in flight we must examine the four main forces which act upon it;
-
Weight is the resultant force of gravity, acting in a downward direction toward the centre of the Earth.
-
Lift is the force that counteracts weight, keeping the missile in flight. Lift is primarily generated through the use of aerofoils.
-
Thrust is the force that propels a missile in the direction of motion. Engines and the combustion of fuel produce thrust.
-
Drag is the force that opposes the direction of motion. Drag is caused by friction and air resistance
IMAGE SHOWING EACH FORCE
​
​
​
​
​
​
​
​
The four main forces which act upon a missile in flight, the video beside it shows an example flight of a missile.
​
The resultant motion and acceleration of a missile in flight can be approximated with reasonable accuracy using these four forces.
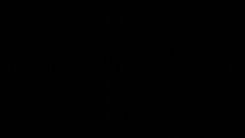
NEWTON'S LAWS OF MOTION
Having considered the origin and direction of each of the four main forces acting upon a missile we now need to examine Newton’s laws of motion to explain their effects.
​
Newton's first law states that a body in a state of motion will remain in uniform motion unless it is acted on by an outside force. This means that if an object is in motion, it will continue with the same velocity until an unbalanced force is applied. When the forces acting on an object are unbalanced, resulting in a ‘net’ force, acceleration of the object will occur. For example, if we were to remove the thrust, drag and lift forces from a missile (possibly when operating at a very high altitude) the downwards force of gravity would accelerate the missile towards the Earth.
​
If we were to eliminate all of the four forces acting upon a missile once it is in motion (in deep space for example) it would continue to move uniformly unless acted on by an additional force.
​
Newton's second law states that the rate of change of momentum (acceleration) of an object is proportional to the force acting upon it, and in the same direction as the force. This means that the acceleration of an object will be greater with larger forces. So a missile which generates greater thrust will experience a faster change of momentum (a greater acceleration). This can be stated as;
​
​
​
​
​
Where F is the force acting on an object, m is its mass, a its acceleration (equivalent to the rate of change of its velocity, v)
​
Newton's third law states that every action has a resultant equal and opposite reaction, meaning that whenever a force is applied to an object there must be a reaction equal in magnitude and opposite in direction to the applied force. If an object is in motion, and we try to change either the direction or rate of that motion, the object will exert an equal and opposite force. That force is directly proportional to the mass of the object, and to the rate of change in its velocity.
​

The Four Forces
THRUST
Our thrust force is a result of Newton’s laws of motion. When an engine pushes or accelerates mass in one direction (the combusted fuel of a missile or rocket) there is a thrust just as large in the opposite direction, following from the third law.
​
DRAG
The opposing drag force occurs due to the same physical principles. Air particles which are deflected and thereby accelerated by (and in the same direction as) the motion of the missile produce an equal and opposite resultant force on the missile, opposing the thrust.
​
WEIGHT
The weight of an object, w is the force of gravity on the object and may be defined as the mass times the acceleration of gravity, w = mg.
​
LIFT
Most missiles, such as the V2 rocket, make use of aerofoils to provide them with a sufficient lift force to counteract the force of gravity and maintain their altitude. An aerofoil is a structure with curved surfaces which are designed to give the most favourable ratio of lift to drag in flight. They are most commonly recognised in the wings of aircraft.
​
​
​
​
​
​
​
​
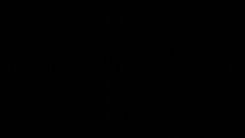
AEROFOILS AND THE PHYSICS OF LIFT
​
​
Examples of aerofoil designs, we will cover more on supersonic flight in the advanced section.
​
There are a number of theories as to how an aerofoil generates lift. The predominant theory is in relation to Bernouilli’s principle. This states that air moving at a higher velocity has a lower pressure. The theory follows that with an aerofoil designed to have a longer path over the top edge than that of the bottom edge (such as the subsonic aerofoil designs in the figure above) air must travel faster in order to pass over the aerofoil in the same amount of time. The resultant difference in pressure generates lift, with higher air pressure below the wing or aerofoil.
​
Bernoulli’s equation concerns the conservation of energy and states the following;
​
​
​
​
​
​
​
This diagram demonstrates how when the fluid (air in our case) moves at a higher velocity we get a lower pressure due to conservation of energy.
​
This theory is partially correct, when the pressure variation is summed across the entire aerofoil body we do determine the lift generated to a reasonable accuracy however we can see in the simulation below that the air passing over the top and bottom edge does not do so in the same amount of time. In addition to this, it does not explain how aircraft can fly upside down and so there must be more physics behind the theories of flight.
​
​
​
​
​
​
​
​
​
Simulation of airflow passing around an aerofoil.
​
In addition to the generation of lift from the difference in air pressure, we can explain a further component of lift if we return to Newton’s laws of motion.
​
From Newton's second law of motion, a force is present when an object is accelerated. If we look at the motion of air particles directly around an aerofoil, they remain in contact with its surface and with the shape of the aerofoil, we end up with a net deflection of the air particles, changing their overall velocity and accelerating them downwards. We know that this acceleration must be due to a force exerted by the wing and that as a result, there must be is an equal and opposite reaction force acting on the wing, a further component of lift.
​
​
​
​
​
​
​
​
​
​
​
​
​
​
Diagram and wind tunnel picture showing the resultant force from the turning flow of air by an aerofoil.
​
For a gas, we have to simultaneously conserve the mass, momentum, and energy in the flow. Newton's laws of motion set out the conservation of momentum and Bernoulli's equation considers the conservation of energy; so both of these principles are satisfied in our explanation of the generation of lift.
​
Although these two theories do a very good job of explaining how an aerofoil generates lift, the real details of flight are very complex. For further reading on the physics of lift click here
​
​
​
​
​
​
​
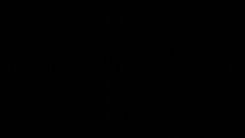
MISSILE GUIDANCE SYSTEMS
The history of guidance systems is short. All of the significant developments are recent, as guidance systems are dependent upon advances in electronics.
​
There are three main types of guidance systems used in missiles;
-
Pre-set Guidance: information to guide missile set before it takes flight
-
Command Guidance: Position of missile regularly determined and signals sent to change its course if necessary.
-
Homing Guidance: Traits of the target are used to track and follow its position.
Pre-set guidance
With pre-set guidance systems, all the information needed to make the missile follow a particular course is set into the missile before it is launched. This information includes the desired heading, altitude, time or length of the flight, and any necessary turns. Once the missile is launched, the guidance cannot be changed although the missile may alter its own altitude etc. based upon what the user has inputted.
​
The missile altitude may be altered by changing the pitch of the missile. In a pre-set guidance system, a barometer may be used to determine the air pressure around the missile and from that determine the altitude at which it is flying. In this way, a particular altitude or pressure can be chosen by the user.
​
The timing component of a pre-set guidance system is one of the most precise parts. He distance covered by a missile is determined by its ground speed and the amount of time it is in flight. If the ground speed is known, the missile can be pre-set to fall at the end of a specific time of flight. The timing component could be a simple watch or for greater accuracy, an electronic circuit with a crystal oscillator.
​
Command Guidance
When command guidance is used, the position of the missile is determined from the ground by radar tracking equipment or similar means. If any error is found between the desired course and the current position of the missile, command signals are sent to the missile to change its course. Command guidance allows corrections to be made for unforeseen circumstances such as changing weather.
​
Radio waves are used in the simplest instances of command guidance. The position of the missile must be determined in relation to the control point, the desired target and the flight path that it should take. Radar is most commonly used to track the position of the missile and target (if it’s moving) and a computer is uses to determine the error between the desired and actual flight path. Signals to change the missiles path are then sent from a radio transmitter at the control point to a receiver on board the missile.
​
Homing Guidance
Homing guidance systems often use some characteristic of the target as a means for tracking, and following it, this is known as passive homing. Almost like a light attracts bugs at night, a missile may follow the bright infrared radiation from an aircraft’s engine for example. Another passive homing system uses radio waves broadcast from the target area as the stimulus to the missile.
​
Infrared homing guidance is common as heat generated by engines or rockets is typically hard to shield. Is not just the heat of the actual engine which can be tracked, heated air is left in the path of the target for some time afterwards. Highly sensitive heat sensors can follow the heated path to the target.
​
The homing system is designed to determine the direction to the infrared source. The missile is then steered in that direction. One way to do this is with two sensors mounted on the missiles with a gap between them. One sensor is able to detect radiation straight ahead and to the right of the missile’s axis and the other is able to detect radiation straight ahead and to the left of the missile’s axis. With this method, when both sensors receive the same amount of radiation, the target is directly ahead. If the radiation received is unbalanced then the target must be out of line with the direction of motion and so the missile can be steered in that direction. In the simplest application this method could be repeated with a second set of sensors controlling the vertical movement of the missile.
​
Other homing systems illuminate the target by radar or other electromagnetic means, and use the signals reflected by the target for homing guidance. This is known as active homing. Usually, a radar set is used and the signals return to the missile after being reflected by the target.
​
As the technology of missiles has developed, their capabilities have been extended. Modern missiles are now capable of flying at ever greater altitudes and speeds, often exceeding the speed of sound. This brings us to the next topic of supersonic flight.
​
The first physical principle we must consider is that of Doppler wavefronts in sound. Any object moving through the air will displace air particles, generating sound waves. Stationary sources produce sound waves at a constant frequency f0 , and the wavefronts propagate symmetrically away from the source at a constant speed v, which is the speed of sound in the medium. The distance between wavefronts is the wavelength and all observers will hear the same frequency, f .
​
​
​
​
​
​
​
​
​
​
​
​
For a source moving at velocity vs, wavefronts are now produced with the same frequency as before, f0 but because the source is moving, the centre of each new wavefront is displaced in the direction of motion. As a result, the wavefronts appear to be closer together in front of the source and more spread out behind the source. An observer in front of the source will now hear a higher frequency f ´ > f0 , and an observer behind the source will hear a lower frequency f ´ < f0 . This is known as the Doppler Effect, seen in action as a car speeds past below.
​
​
​
​
​
​
​
​
The change in frequency, at non-relativistic speeds, is determined with the Doppler equation;
​
​
where c is the velocity of sound in the medium (the velocity of sound in our case), v0 is the velocity of the observer (0 in our case) and vs is the velocity of the source.
​
If the velocity of the source equals the speed of sound (vs = c) the wave fronts cannot escape the source. The resulting pile of waves forms a large amplitude ‘sound barrier’ as seen below.
​
​
​
​
​
​
​
​
​
​
​
​
​
If the velocity of the source exceeds the velocity of sound (vs > c) then the wave fronts fall behind the source in a cone-shaped region known as a ‘mach cone’. This cone is what creates the shock wave and ‘sonic’ boom associated with supersonic flight. When the shock wave reaches an observer a "sonic boom" is heard. An example of this can be seen below as a jet breaks the sound barrier.
​
​
​
​
​
​
​
​
​
​
​
​
​
Unlike typical sound waves, the velocity of a shock wave is proportional to its amplitude. Its velocity is always greater than the speed of sound in the fluid and decreases as the amplitude of the wave decreases. When the shock wave velocity eventually falls to equal the speed of sound in the fluid, the shock wave becomes an ordinary sound wave.
​
The ratio of the speed of a moving object (v) to the speed of sound (c) in a fluid is known as the Mach number (M), after Ernst Mach An object’s Mach number is the most common way to define how fast it is moving relative to the speed sound, and it is defined as;
​
​
​
​
.
Mach 0.5 would be half the speed of sound, whilst Mach 2.0 is twice the speed of sound. At sea level, the speed of sound in air is approximately 340 m/s.
At supersonic speeds, the drag on an object increases exponentially. Missiles designed to fly at these very high speeds must be designed to be very sleek and narrow in order to minimise the effects of drag. The lift generated by an aerofoil increases with speed so only small aerofoils are required for missiles in supersonics flight. Another tactic to reduce drag is to design missiles which operate at very high altitude, where air is less dense.
​
The generation of lift by aerofoils moving at supersonic speed differs from that of subsonic flight due to the shock waves we have discussed. Below is a typical aerofoil used in supersonic flight, we can see the two points at the leading and trailing tip of the aerofoil where shock waves are produced and the resultant areas of compression and expansion.
​
​
​
​
​
​
​
​
​
​
​
​
​
​
​
At supersonic speeds, lift is still generated by a pressure difference, but this difference occurs in a different way to Bernoulli’s principle at subsonic speeds. The pressure is increased in the shockwaves and decreased in the expansion wave. These waves form at an angle relative to the wing and so, as in the picture above, will vary in their angle relative to the air flow.
​
With the aerofoil at a positive angle of attack, as seen above, we get less of a difference in angle between the shock wave and air flow over the wing compared to that of the shock wave below the wing. The result of this is lower air pressure above the aerofoil than below, as in subsonic flight, thereby generating lift. This is easier to picture with the video below, showing the high density region below the leading edge of the aerofoil.
​
​
​
​
​
​
​
​
​
​
​
​
​
​
​
​
​
​
​
​
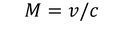

​
Aerofoils at Supersonic Speeds
0
